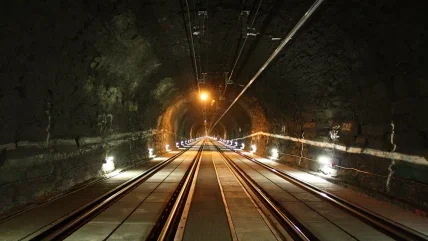
INTRODUCTION
Being part of the global trend of digitalisation, the past decade has seen a rapid increase in the interest in digital techniques for geotechnics and tunnelling. Developments range from augmented reality, an increased use of scanning technology (laser-scanning/ photogram- metry) and artificial intelligence to applications of robots and unmanned aerial vehicles inside tunnels (Marcher et al., 2020; Huang et al., 2021).
One of these topics is building information modelling (BIM) and, for example, Borrmann et al. (2019) define it as “… a comprehensive digital representation of a built facility with great information depth. It typically includes the three-dimensional geometry of the building components at a defined level of detail. In addition, it also comprises non-physical objects, such as spaces and zones, a hierarchical project structure or schedules….”.
BIM is, therefore, a way of modelling buildings digitally before their construction to detect flaws as soon as possible.
While the development of BIM is already far advanced in structural engineering, BIM in geotechnics and tunnelling is currently seeing a rapid development and a transition from academic and pilot use cases to ‘real world’ applications and common practice.
Despite general publications on BIM in tunnelling (Daller et al., 2016; Berdigylyjov and Popa, 2019; DAUB, 2019, 2020; Kapogiannis and Mlilo, 2020; Huang et al., 2021; Ninic et al., 2021), several recent publications address specific aspects of BIM in tunnelling, such as ‘BIM to FEM’ approaches (Alsahly et al., 2020; Fabozzi et al., 2021), settlements (Providakis et al., 2020, Providakis et al., 2021) and others (Ninic et al., 2020). Published case studies on applications of BIM for tunnelling projects are for example Cudrigh-Maislinger et al. (2020); Weichenberger et al. (2020); Wenighofer et al. (2020); Mitelman and Gurevich (2021); Wang and Zhang (2021).
While there is extensive literature on different aspects of (engineering) geological 3D models from past decades (e.g., Gong et al. (2004); Caumon et al. (2009); Horner et al. (2016); Erharter et al. (2021)), the specific literature about BIM ground models (BIMGM) is rather sparse with few examples (Kessler et al., 2015; Daller et al., 2016; Weil, 2020). The referenced publications mostly address specific technical tunnel-related topics, while this paper will focus on the geotechnical ground model as a part of the overall BIM coordination model of a tunnelling project.
We first present background information on the state of the art and current developments in BIM ground modelling on which the presented concepts are based (Section 2).
In accordance with these developments, we then contextualise the BIMGM within a construction project’s overall BIM structure and also within a project’s life cycle (Section 3). The section also addresses different possible use cases of the BIMGM.
In Section 4, we present the concepts of selected ‘sub-discipline models’ of a BIMGM which are then applied to the real-world case study – Angath Tunnel’, in Section 5.
The paper closes, in Section 6, with a discussion of the presented BIM framework, its limitations and an outlook to future developments. Information for supplementary material can be found in the end of the paper, where an example BIMGM is included as an .ifc file with a corresponding exemplary attribute list.
The concepts about BIM ground modelling discussed here were developed in the context of the project described and other real-world projects, and also the authors’ involvement in two working groups on BIM ground modelling (DAUB and IFC Tunnel).
The rapid development of BIM in the past years has produced a vast amount of (sometimes redundant or even conflicting) terminologies and definitions. While this paper aims at using consistent and clear terminology, including an extensive glossary of definitions would be out of the scope of this study. References to synonymous terms are given throughout the text, but the reader is for example referred to Borrmann et al. (2019, pp.575–578) for general BIM glossaries and to DAUB (2019, pp.40–41); buildingSMART (2020, pp. 51–52); Molzahn et al. (2021) for BIM ground modelling/ tunnelling-specific glossaries and terminology definitions.
2. BACKGROUND
2.1 State of the art
Digital ground models became state of the art in infrastructure projects in the last decade, as an additional or alternative way to represent ground conditions. Databases and digital data exchange formats replace hardcopy documentation of factual data, i.e., observations and measurements that describe the conditions at certain locations.
Several countries established a common practice and standardised formats, e.g., AGS (Bland et al., 2014) in Great Britain and several other countries or DIGGS (Cadden and Keelor, 2017) in the US. Conceptual data models, e.g., by OGC (GeoSciML (OGC, 2017), GroundWaterML (OGC, 2021)) are frequently used in national geological surveys, infrastructure owners or other larger organisations that need to work with extensive geoscience-related datasets. The general application of ‘Engineering Geological Models’ has recently been described by a guideline of the IAEG Commission 25 (see Baynes and Parry (2022) and Parry et al. (2014)) and can include digital 3D models (observational models), describing the expected distribution of relevant aspects in the model space, based on geological conceptual models and are used to derive geotechnical models for specific use cases. Traditionally, these models were described by reports, maps and sections which can now be linked to and extracted from digital models.
Nevertheless, there are no commonly agreed standards for the digital exchange of geological and geotechnical data in infrastructure projects.
In the last two decades, 3D ground models were implemented as a helpful and efficient tool, and many examples around the world are described in literature, e.g., for alpine tunnels (Cudrigh-Maislinger, 2018), metro projects (Huang et al., 2022) and hydropower (Weil et al., 2019), and further developed in ongoing research projects (e.g., Gächter et al. (2021)). However, solutions and data structures for these projects were mainly developed independently by their authors and model users and do not follow detailed definitions of requirements by project owners. This implies frequently enormous efforts for digitalisation, transformation and mapping of information between different formats and software.
In the DACH countries (Germany (D), Austria (A), Switzerland (CH)), the BIM method has been applied on many infrastructure projects, being pushed by government agencies (BMVI, 2022) and large infrastructure owners, with the intention to establish the method as ‘common practice’. Even though ground models were not a main focus in most cases, this development triggered a transition towards digital methods and 3D modelling being applied in the field of engineering geology and geotechnics.
With both the BIM method and digital ground models becoming more accepted, the demand for standardisation and clear definitions has become obvious. Many national and international working groups have formed to address these requirements and publish recommendations. Several initiatives are mentioned below, selected either because the authors are personally involved or their output was considered in the approach used in the tunnel project case study here discussed.
2.2 Current developments and standardisation
All initiatives described below started with definitions of requirements and have different backgrounds: From focus on specific needs in tunnelling (DAUB, IFC Tunnel), over the general field of geotechnics and earthworks (DGGT, IFC common schema covering geo- technics in IFC 4×3), to a more global scope considering interfaces to mining and resources, oils and gas, environmental and other geosciences (OGC).
Working groups are formed by participants from federal agencies, infrastructure owners, consultants, contractors and software developers. The developed concepts vary from more general description of model structures, object catalogues and example property sets to detailed data models (e.g., in UML) and definition of extensive attribute lists representing the definitions in national, EN and ISO standards for ground characterisation and geotechnical testing.
- The German branch of ITA released recommendations for BIM in tunnelling (DAUB, 2019) and model requirements (DAUB, 2020) that have been welladopted by the industry. The latest recommendations in this series was published in autumn 2022 and covers geotechnics and ground models, with definitions of use cases, model structure and typical property sets elaborated by a working group of geologists, geotechnical- and tunnel engineers (DAUB, 2022). The model structure described below (see Section 4) was developed based on work within this group.
- The German DGGT published detailed recommendations and concepts for model structures of geotechnical models. Data catalogues with definition of attributes and property sets have been published recently (DGGT, 2022), covering both factual and interpreted models (homogeneous areas (German: ‘Homogenbereiche’) according to (DVA, 2016))
- The IFC standard format for the exchange of BIM models in OpenBIM environments is currently being extended to infrastructure models (buildingSMART, 2022), including tunnels. The latest released version IFC 4×3 already included a simple schema for interpreted geotechnical models and boreholes. In the course of the development of conceptual models for IFC Tunnel, an extension of this schema is planned to cover factual data (observations, measurements) with links to existing exchange formats for factual data as well as different types of interpreted models, several concepts to treat uncertainty in modelling, and an extension of the IFC format to realise voxel representations. A focus is on the concepts to link the definition of expected ground conditions to the tunnel alignment/ design model as realised in the example model (see Section 4). The German section of buildingSMART recently published literature and a similar schema in German language (Holsmölle, 2022).
- The Open Geospatial Consortium (OGC) just launched an initiative to extend its schemas to geotechnical models (OGC, 2022), mainly driven by the French MINnD group. This initiative is coordinated with buildingSMART international and intends to maintain a common concept for the geology/geotechnics domain that is implemented by both IFC and OGC standards in future. In addition, a collaboration with the International Society for Soil Mechanics and Geotechnical Engineering (ISSMGE) is expected. ISSMGE recently formed Technical Committee 222 (Geotechnical BIM and Digital Twins) as a forum for members “to disseminate and exchange knowledge and practice” on BIM and DT in geotechnics (ISSMGE, 2022).
Based on published materials and discussions, it can be concluded that the above-mentioned groups developed similar concepts for ground models, even though differences in terminology, focus and presentation of the results exist.
3. CONTEXTUALISATION OF THE BIM GROUND MODEL
Whereas the previous section presented background literature, state of the art industry applications and government guidelines, this section sets the BIMGM into a hierarchical context within a project’s bigger BIM model structure (see Section 3.1) and within a building’s life cycle (Section 3.2). Based on this, typical expectations for a BIMGM from the client’s perspective are defined (Section 3.3).
3.1 Integration within the overall BIM structure
Following the proposed structure by DAUB (2020), the BIMGM is a ‘discipline model’ and “contains specific information from the single specialist designer in charge of their discipline”. (This discipline model corresponds to the ‘Partial Model’ of Borrmann et al. (2019)).
It has to be noted, however, that a ‘BIM discipline model’ does not necessarily contain all the available information but is rather a filtered and specifically prepared model to be integrated in the overall BIM (coordination) model. This can be compared to typical plan preparation, where finalised plan documentation does not contain all ‘working data’ that were necessary and gathered to enable their creation. The working model that is used by modelling personnel would only be called the ‘domain model’ and it is usually not included in the final BIM model. The amount of information that goes from the domain model to the ‘BIM discipline model’ must be defined individually for each project, based on necessary requirements of all involved parties and the long-term intended application of the BIM model.
The BIMGM is one of the discipline models in a tunnelling project, such as excavation and support and systems. Hierarchically, the discipline model is located below the ‘Coordination Model’ and above the ‘Object Groups’ (Figure 1).
The discipline model itself can be subdivided into ‘sub-discipline models’, which refers to a separation into contextually specific models (e.g., a factual data model, a geotechnical model, a hydrogeological model, etc.,) and into ‘sub models’, which refers to geographically separated parts of one sub-discipline model (e.g., multiple sub-models for multiple construction lots of one bigger project).
3.2. The BIM ground model within the building life cycle
The BIMGM is subjected to constant change and update throughout a tunnel’s life cycle and consequently also serves different use cases. Use cases in the design phase of a tunnel are, for example: compilation of existing data and knowledge within one model; route selection; visualisation and communication of complex ground conditions; coordination of different disciplines that address ground-related topics; estimation of excavation quantities; providing input for geotechnical assessments (e. g., numerical modelling); preparation of tender documents.
However, the BIMGM is not only a tool for the design phase of a project but should also serve different purposes throughout the rest of its life cycle. During construction, the BIMGM can be further used as a database for the collection and combination of the digital geological-geotechnical documentation from the excavation. It enables model-based comparison of expected versus encountered conditions with a ‘single source of information’ and thus increases the efficiency of keeping an overview of the excavation progress.
During the maintenance and operational phase, both a BIMGM from the planning phase and one that documents the ‘as-built’ state from the construction phase should be available to help identify possible ground-related damages that can occur with a long time lag (e.g., long-term settlements, fractures, unforeseen water ingress, etc.,). A future use case of BIMGMs is discussed in Erharter et al. (2022), which concerns knowledge derivation for future projects based on bigger BIM databases. Several of the cases are based on DAUB (2022). A graphical representation of use cases of the BIMGM throughout a building life cycle is given in Figure 2.
3.3. Expectations for the BIM ground model
The basic requirement for the BIMGM is that its information content (geometrical and metadata) is at least the same as in conventional/ non BIM-based planning and in accordance with all current standards and guidelines.
BIM-based planning should not be an end in itself but to create an actual benefit for the tunnel.
The main purpose of the BIMGM in the planning phase of a tunnel is to ease the communication between different parties as it can serve as a central source of geological information. Based on that, everyone should be able to derive information as desired; lengthy coordination between planning companies is needed to avoid generation of error-prone plans (a discussion on the current limitations of visualisation of geological data is given in Section 6).
Although geological 3D modelling has been done for several decades, the rise of BIM has pushed geological and geotechnical 3D modelling in civil engineering. Therefore, BIM ground modelling is often expected to increase the quality of the geological prognosis. In reality, the geological 3D modelling and 3D visualisation of buildings within the geology help all involved parties to get a better understanding of complex conditions and thus increase the value of the geological model.
It must be noted that geological 3D modelling can only improve the previously mentioned aspects of a geological prognosis, and it is as dependent on the quality of the engineering geological investigation as conventional geological planning.
In the context of modern tunnel construction contracts, like Design and Build or Alliance (Deutschmann, 2021; Karasek, 2021), BIM-based planning has the advantage that it can help to communicate the totality of complex ground conditions better to potential contractors than done by classical 2D-based planning. Geotechnical baseline conditions and the included uncertainty/expected variations described, e.g., in a Geotechnical Baseline Report (GBR), according to FIDIC Emerald book can be represented in a ground model.
By delivering a BIMGM as well as domain models (native formats and software, as discussed earlier in Section 3.1 and Figure 1), the complete geotechnical and geological information can be included but also represented in a manageable format.
4. BIM GROUND MODEL: SUB-DISCIPLINE MODELS
4.1. Overview
As discussed in Section 3.1, the BIMGM can be separated into several sub- discipline models. In this section, three such sub-discipline models are presented: the Factual Data Model; the Geotechnical Model; and, the Geotechnical Synthesis Model.
The authors note that the three sub-discipline models do not represent the overall best approach to a BIMGM but were created in the planning phase of the case study of Angath Tunnel (discussed in Section 5.) Further, the authors add that while the approach fitted the requirements of that case study, other projects might call for a different choice of sub-discipline models.
The basic distinction between ‘not-interpreted’ or ‘factual’ models (here, the Factual Data Model) and ‘interpreted’ models is supported by all working groups referred to earlier, and is in line with the classical approach of data exchange and reporting (e.g., Geotechnical Data Report versus Geotechnical Interpretive Report and GBR, according to FIDIC Emerald Book (FIDIC, 2019)).
In addition to the sub-discipline models mentioned, several others – especially interpreted models – are conceivable in the context of BIM ground modelling (e.g., a hydrogeological model, material recycling model, etc.,). For example, it is recommended to have a geological model ready before a geotechnical model is created (Parry et al., 2014), but in the project reality it is not always necessary to implement each sub-discipline model as a BIM model (even though it may exist in the native domain model).
A BIMGM must always be accompanied by a geotechnical report that describes its purpose, concept, modelling approach and the classification that is represented by it. It can either replace other documents, like longitudinal- or cross-sections, or can be used to extract them. Technical details of the implementation of the BIMGM should be given in the BIM Execution Plan (BEP).
In this paper’s supplementary material, an exemplary BIMGM is given as an .ifc file (IFC 4X1) including all three sub-discipline models (Factual Data Model, Geotechnical Model, and the Geotechnical Synthesis Model). The BIMGM is accompanied by an exemplary list of model objects and attributes, defining the BIMGM’s semantics as given in the beginning of a project in the BEP. An overview visualisation of the exemplary BIMGM is given in Figure 3 and further information on this is given in the subsequent subsections and Section 5. The content of the exemplary model is only for representative purpose and not directly based on the real BIMGM of Angath Tunnel.
4.2. Factual Data Model
The Factual Data Model represents the BIM version of the content of a ‘Geotechnical Data Report’. In the planning phase of a project, the model contains the information that has been collected during site investigation (e.g., borehole data, results from in-situ and lab testing, geophysical investigations, documented outcrops, etc.,). During construction, the scope of the Factual Data Model is then extended to serve as a database for documented geotechnical observations (e.g., tunnel face mapping, collected samples from the investigation, etc.,).
The exact line between ‘factual’ and ‘interpretation’ is not clear, as any description of natural material implies a certain degree of interpretation. The same applies to deriving geotechnical parameters from measurements during tests. In this context, the limit is usually set according to data exchange points, like information submitted from a laboratory, contractor for drilling and in-situ testing, or a field geologist.
4.3. Geotechnical Model
In contrast to the Factual Data Model, the Geotechnical Model is an ‘interpreted’ sub-discipline model as its content is fully based on processed information from the former and also interpolation between points of confirmed information.
The Geotechnical Model is derived from an engineering geological conceptual model for a specific purpose, like e.g., tunnel design in a certain project phase. It represents volumes of homogeneous properties which are called geotechnical units that can be separated from each other with volumes or with boundary surfaces only. In the latter case, however, it has to be specified exactly if the boundary surfaces represent upper or lower boundaries for the respective geotechnical unit (see also buildingSMART (2020, p.67)).
In cases of high investigation densities, well-known ground conditions and/ or high-resolution models, the attributes of the geotechnical units can comprise specific information concerning, for example, the general geology (geological formation, lithological characterisation, etc.,) or mechanical properties of the ground (e.g., density, friction angle, cohesion etc.).
In cases of deep tunnels, where an exact localisation of geotechnical properties is often not possible (ÖGG, 2021), a high degree of uncertainty and/ or a generally low resolution model has to be produced. The geotechnical units’ properties then can be made up of distributions of ground classifications (e.g., rock mass types, Q-classes, etc.).
In Figure 4, the concept of the geotechnical model with multiple geotechnical units is visualised and also two examples of attributes are given.
Uncertainty related to geological investigations or interpretations can be represented within the model either via attributes (i.e., qualitative or quantitative description) or also via the geometry itself by, e.g., modelling different likelihoods of boundary surfaces between geological units. Dealing with the topic of uncertainty itself is, however, in principle not different for BIM ground modelling in comparison to conventional engineering geological modelling (Weil, 2020).
4.4. Geotechnical Synthesis Model
The Geotechnical Synthesis Model contains all the 1D information along the tunnel that is usually communicated with longitudinal sections (Figure 5). The geometry of it can be as simple as a tube along the tunnel axis, that is split into multiple sections of homogeneous properties.
The name Geotechnical Synthesis Model was developed in the IFC Tunnel working group as a term used for a sub-model that contains the essence of the other sub-models in relation to certain buildings or design structures, like e.g., a tunnel tube and its alignment. Developed during the design phase of a tunnel, it is used as an interface to the model of the planned building, especially the planned excavation and support structures that interact with the ground.
One important function is to consider uncertainty in prediction of geological structures and geotechnical conditions to be expected along the alignment. It follows a common approach in tunnelling: the definition of ‘homogeneous sections’ along the alignment with characteristic, similar geotechnical conditions and expected ground behaviour, usually defined for certain chainage intervals and documented in a longitudinal section (Figure 5). Such planning documents are commonly used as the basis for defining and/selecting the choice of excavation methods, support types, ground improvement and other measures, as required, and also estimation of quantities, advance rates, etc. This is a common way to document the contractual basis of expected ground conditions.
The intervals of the Geotech Synthesis Model quantify or rate the relevant geotechnical aspects. These aspects can be described in the other sub-discipline models (geological, geotechnical, hydrogeological models) which allows extraction of relevant information. This can comprise:
- expected distribution of geotechnical ground types
- discontinuity properties
- groundwater conditions
- geogenic hazards, contaminations and other aspects
Based on this condensed information with reference to the tunnel, the designer can define solutions and ‘answer’ with a design-prognosis model, including:
- planned excavation methods
- expected distribution of support types
- expected additional measures for ground improvement, health and safety, logistics, etc.
Explicit geometric modelling of complex geological structures, such as discrete fracture networks (see e.g. Pan et al. (2019)), can be valuable for specific use cases (e.g., finite element modelling (FEM)) with high resolution models for local-scale building structures and surrounding ground.
For long and large buildings, like kilometres of tunnel, it is considered more appropriate to include the information on typical discontinuity orientation and conditions in the attributes of the model. For this reason, discontinuities like joints and faults are characterised in the subject example model by special attributes of the Geotechnical Synthesis Model, describing the typically expected conditions for each individual tunnel section.
5. CASE STUDY: ANGATH TUNNEL
In this section, implementation of the BIM concepts discussed above is presented for a project case study, concerning the BIMGM of the Angath Tunnel, located in the Lower Inn Valley, in the Austrian federal state of Tyrol. The rail tunnel project runs through the ‘Unterangerberg’, which is a smooth plateau that rises around 150m above the valley floor and has a maximum elevation of ~ 680m a.s.l.. The River Inn confines the Unterangerberg to the South and the nearest city to the project is Wörgl.
The Angath Tunnel is on the Schaftenau – Radfeld section of the trans-Europe rail line being developed in stages to connect Berlin to Palermo, and is also part of the northern access to the Brenner Base Tunnel. Within this route section, Angath Tunnel is a side tunnel located to the south and parallel to the main rail tunnel alignment and it is to be constructed in advance of the main tunnel tube. They are to be connected by six cross passages. A special access road to the construction site has to be built.
An overview of the tunnel project, the BIMGM model extent (see subsection 5.2) and locations of nearby exploratory drillings are given in Figure 6.
5.1. Engineering geological overview
The Unterangerberg comprises geological conditions that are demanding for both the planning phase (Erharter et al., 2019) and for the excavation. The tunnel will be excavated in the bedrock of the Unterangerberg Formation (UAFm), which features a rugged bedrock surface with pronounced WSW-ENE striking gullies and ridges that are covered by glacial sediments (Poscher et al., 2008; Sommer et al., 2019).
The UAFm is part of the ‘inneralpine Molasse’ and is made of prodelta sediments, deposited into marine basins affected by synsedimentary deformation (Ortner and Stingl, 2001; Ortner, 2003). The lithology of the UAFm consists of alternating claystone/marl and sandstone layers of thicknesses varying between a few millimetres to several centimetres. The rock is therefore highly anisotropic due to the low uniaxial compressive strength of around 25MPa. It can be classified as a ‘hard soil – soft rock’ material (Kanji, 2014). The rock mass of the Unterangerberg is intersected by several zones of tectonic disturbance with increased occurrence of fault zones. Located between the bedrock and the glacial cover is a zone of heterogeneous weathering up to 35m thick (Erharter et al., 2019).
The cover of glacial sediments above the UAFm consists mostly of fine to coarse grained glaciofluvial to glaciolacustrine deposits (Ilyashuk et al., 2022). In the portal area there are mostly fluviial sediments of the Inn Valley, consisting of sandy to gravely river deposits under a layer of topsoil with high organic content. The portal is also located near highway A12 Inntalautobahn, which is why extensive anthropogenic deposits and remodelling of the landscape is expected in this area.
5.2. STRUCTURE OF BIM GROUND MODEL
In order to meet different use cases of the BIMGM, three sub-discipline models (see Section 4). An overview of the models is shown in Figure 7.
For the 3D modelling and attribution of the BIM elements of this case study, a combination of different software packages was used (see Table 1). Similar ground models can be created with alternative software products on the market and commonly used by the industry.
Export and data exchange are done in the .ifc data format in the latest available version at the time of modelling (IFC 4×1). Since there are no suitable classes (BIM Types) for ground modelling yet, all objects are classified as ‘BuildingElementProxy’. To still be able to have some classification of the object types and to ensure more clarity in the IFC viewers when examining the BIMGM, and to differ between the three subdiscipline models, the objects have been assigned to ‘buildings’ and ‘stories’ similar to structural engineering projects. Three ‘buildings’ were created for the three sub-discipline models, as seen in Figure 7, with the individual objects assigned to their own ‘stories’/classes.
Table 2 shows the applied allocation of the ‘story’/ class (for the Classification System) to the corresponding ‘building’. It must be noted that this is a workaround, as a result of the current state of technology that does not allow for creation of custom classes/ object types.
Some IFC viewers allow additional sorting by using classification systems. In this case study, for the Angath Tunnel project, a user defined classification system was created with classes analogue to the previously defined ‘stories’ and each object was assigned a class. An exemplary BIMGM with the described classification methods can be found in the supplementary material of this paper.
The three sub-discipline models of the BIMGM consist of objects that are modelled from geometries with associated attributes. To ensure that the information content/ semantics of the BIMGM are fully understandable for other involved companies, an attribute list was developed from the start of the project that assigns attributes to each object. These attributes were created as user defined PSets with the data modelling function of BricsCAD BIM. A simplified attribute sheet for exemplary purposes also can be found in the supplementary material.
To fulfill all requests from the client and other planning companies, it was agreed that the BIMGM should horizontally cover an area of at least 60m around Angath Tunnel, the connecting tunnels and the access route to the construction site (see red dashed line in Figure 6). The lower boundary of the BIMGM was set to 440m a.s.l. as the lowest point of the tunnel is at 473.75m and it was the goal to have the model extend at least 30m below its lowest point. Considering a possible application of the BIMGM for numerical/ FE modelling, the model extent was based on the suggestions of EANG (2014); it was recommended to have a model extent around the tunnel of 4–5 tunnel diameters horizontally and 2–3 tunnel diameters vertically.
5.3. Sub discipline models
For the Geotechnical Model, homogeneous areas with the same geotechnical properties were modelled as 3D volumes. The volumes are provided with the percentage distribution of individual rock mass types (see Section 4.3) as well as further geological information. For each volume, five rock mass types with associated attributes can be defined.
The following attributes can be added to each rock mass type in the Geotechnical Model:
- Identification of the rock mass type
- Volumetric percentage of the rock mass within the volume
- Associated description
- Link to a data sheet with further geotechnical parameters
To link the geotechnical units of the geotechnical model with associated data sheets of the rock mass units, relative paths were given in the attributes of the volumes.
The datatype of these relative paths is a ‘string’, but – depending on the used IFC viewer – the paths can be directly used to access further .pdf files from the BIM model. To create these, relative paths are inserted starting from the location of the IFC file to linked files or folder paths.
An example for a relative path to a linked drill log is shown in Figure 8. In addition, an example of how to access a superordinate folder is shown.
Figure 9 shows an exploded view of the Geotechnical Model, which consists of 17 volumes that represent individual geotechnical units of Angath Tunnel. The topmost geotechnical units comprise the following sediments: anthropogenically modified sediments; topsoil and deposits from the River Inn; quaternary cover of the Angerberg (yellow, white and orange colours, respectively, in Figure 9 and Figure 10). Below these, several geotechnical units represent either weathered or unweathered Unterangerberg Formation (purple and dark-purple, respectively) or different zones that have been subject to tectonic deformation (orange).
Additionally, 10 cross sections were generated to provide a convenient view for pre-defined longitudinal, transverse or horizontal sections through the tunnel. In the attributes of the cross sections, relative paths to 2D section plans are added.
According to Section 4.4, the Geotechnical Synthesis Model is the representation of a classical tunnel longitudinal section. For the representation, a segmented 3D model is established with a simplified, representative tunnel shape. In individual tunnel sections, information about gas or water ingress, interface information, etc. are represented.
If varying information (e.g., A, B, C, D from Figure 5) are combined within one Geotechnical Synthesis Model, many small sections result. To avoid excessively small and complex sections, several models are created for the different data. Doing this is a trade-off between having few models with maximum of information content versus the user friendliness and readability of the whole Geotechnical Synthesis Model, which is increased if there are multiple models for different categories of information.
Five such models were created for Angath Tunnel:
- Geology: consisting of nine sections with distributions of different rock mass types.
- Discontinuities: consisting of four sections with the prediction of discontinuity properties such as their orientation.
- Water: consisting of 12 sections with water ingress forecasts and predicted water pressures.
- Gas: consisting of three sections with forecasts of gas inflows.
- Swelling: consisting of two sections with forecasts of swelling phenomena.
In the respective Geotechnical Synthesis Models, different attributes are added depending on the type of information. For example, the type, azimuth and angle of interfaces are defined in the model ‘discontinuities’.
The Factual Data Model represents uninterpreted facts, as discussed in Section 4.2.
For the Angath Tunnel, 36 boreholes were modelled. For better visibility, the boreholes were modelled as cylinders of 1m-diameter. In the attributes, further information such as the start and end of drilling, water level and a link to drilling log files were given. Figure 10 shows a rendering of the BIMGM with all three subdiscipline models. The volumes of the Geotechnical Model correspond to the exploded view that is given in Figure 9.
6. CONCLUSION AND OUTLOOK
A new concept of BIM ground modelling has been presented that is based on – and in accordance with – recent developments from different international working groups. The BIMGM of the Angath Tunnel was discussed as a real-world case study of implementation of the concepts, which worked perfectly fine and the chosen software pipeline proved to be sufficiently versatile and flexible.
The authors wish to emphasise that the concepts and their implementation should be understood to be only a ‘snap-shot’ of the currently ongoing and fast developments in this field. But the paper should also serve as a guideline and example to ease entry into the world of BIM ground modelling for practitioners with limited BIM experience.
Despite many efforts to push the boundaries of BIM ground modelling, several topics can be seen as limitations of the current approach and need to be improved upon.
While there are first attempts to establish the concept of the Level of Development (LOD – see for example Borrmann et al. (2019, p.10)) for the tunnel (DAUB, 2019), there are currently no accepted standards that propose a LOD for the ground model itself. DAUB (2022) presents certain granularity levels for ground modelling but this also has yet to prove its practicability. This deficit might be connected to the comparably high degree of uncertainty in ground models and also to the fact that every ground model is unique and project specific. Nevertheless, general guidelines on this topic are desirable from a client’s perspective (for clear and assessable calls in BIMGM bids) but also from the perspective of the modelling company.
Another challenge is seen in the IFC format for ground modelling. In the current state of IFC, custom properties have to be manually defined for every project which is a process that is both laborious and error prone. Furthermore, BIMGMs that are saved as IFC files are not usable for further modelling as few geological/ geotechnical software packages can deal with it directly but rely on their own proprietary file formats. This is also problematic when BIM models should be connected to Geographical Information Systems (GIS), which are widely used in geology and the infrastructure sector but hardly ever fit for BIM implementations.
Considering the (often highly praised) application of BIM models throughout the whole life cycle of buildings (Section 3.2), it is a special question for infrastructure projects (with long planned lifetimes, of more than 100 years) whether models from the planning phase would be usable several decades later when perhaps longterm – possibly ground-related – damages may have occurred on the building.
Easy visualisation of geological information on a BIMGM is a challenge today. Although a BIMGM can contain the exact same information as a conventional 2D geological tunnel section, the information on the 2D section is more eye-catching and easily readable; information in the BIMGM must be looked for and found.
This might be a minor visualisation problem but could be the source of miscommunication and errors. It could be addressed with more advanced viewing technology that is specialised on displaying tunnel-related geological information.
Another challenge is the necessary level of software skills that BIM ground modelling demands. Standard university education hardly ever involves applied courses on geological 3D modelling, or even BIM modelling. The demand on the personnel is therefore increasing as knowledge of a whole set of software packages and possibly different programming languages is required to produce state of the art BIMGMs. The case study of Angath Tunnel demonstrates well how one has to be able to establish a whole software pipeline as there is not one single software program that can fulfill all requirements.
Whereas current developments of BIM for ground modelling often focus on the planning phase (as in this study), the coming years will show how well BIMGMs can be integrated into the construction phase of a project. It is yet to be proven that economic benefits arise when BIM models are used for automatic ‘asplanned’ versus ‘as-built’ comparisons, and that the model of the ‘planned geology’ can be easily updated with information from the excavation.
Lastly, it should be noted that although there are several needs for improvement, BIM ground modelling has benefits for the whole field of geotechnics as it forces people to establish standardised procedures for activities that, for many years, have been solved by ‘in-house’ or custom solutions. The high degree of standardisation that BIM demands should be seen as an opportunity to make geotechnics more transparent and comprehensible and thus improve the industry’s standards as a whole.
Declaration of Competing Interest
The authors declare that they have no known competing financial interests or personal relationships that could have appeared to influence the work reported in this paper.
Data availability
A link to the data was shared at the Attach Files step and within the Supplementary material section of the paper.
Appendix A. Supplementary data
Attribute_list.pdf and BIMGM.ifc can be downloaded from: Link to TU Graz Repository (https://doi. org/10.3217/007z6-7sh07)
Open Access
This is a slightly abridged and. Edited version of the full paper published online in Tunnelling and Underground Space Technology (incorporating Trenchless Technology Research) 135 (2023) 105039, under a Creative Commons (CC) licence. https://doi.org/10.1016/j.tust.2023.105039 Received 25 July 2022; Received in revised form 9 January 2023; Accepted 5 February 2023, Available online 15 February 2023